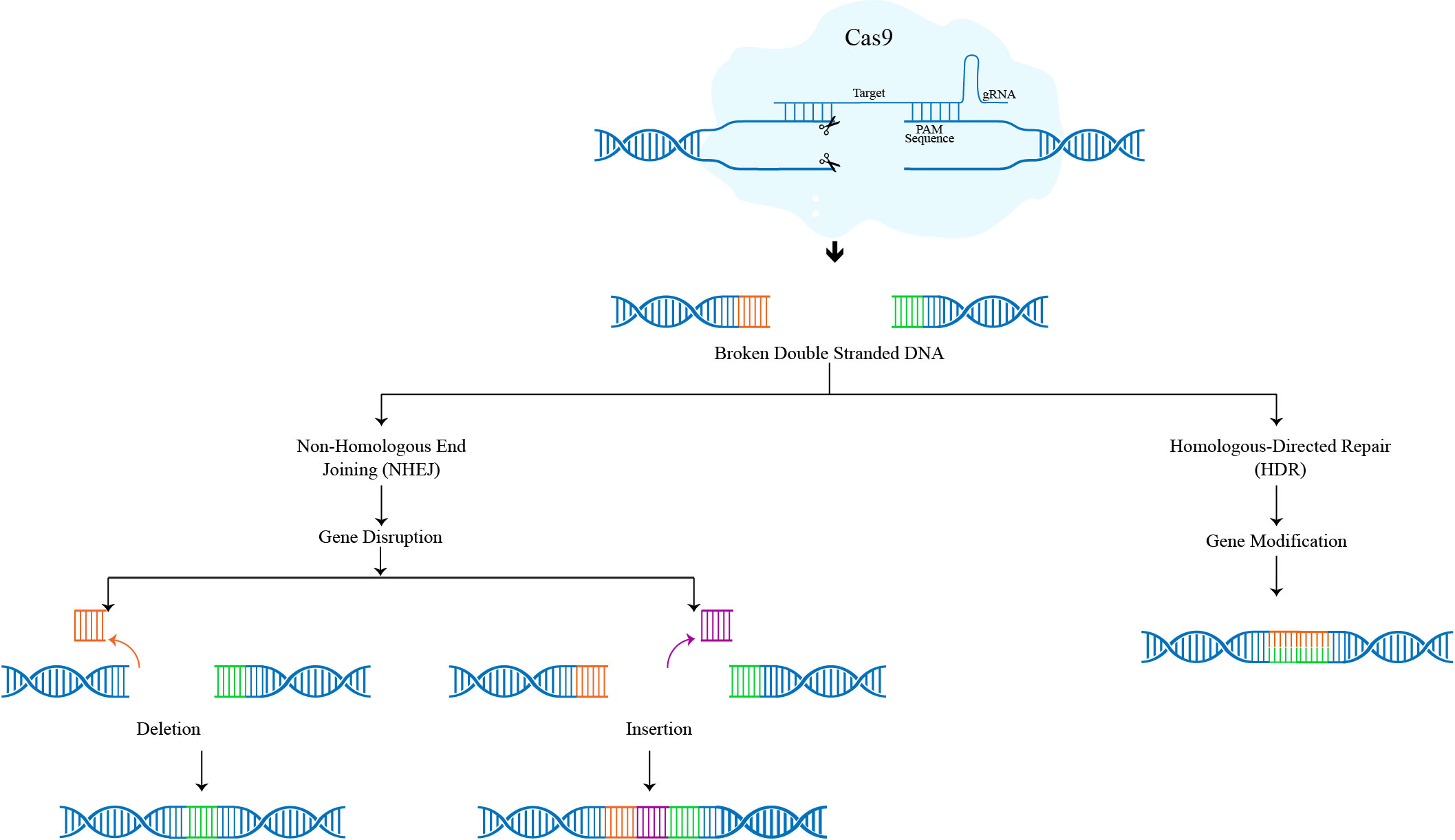
Role of CRISPR-Cas9 in Genome Editing
Genome editing, also known as gene editing, refers to a range of scientific techniques that enable the modification of an organism's DNA. At specific sites in the genome, these technologies help in the addition, removal, or modification of genetic material. Several approaches for genome editing have already been developed. CRISPER-Cas9, which stands for clustered regularly interspaced short palindromic repeats and CRISPR-associated protein 9, is a well-known example. The CRISPR-Cas9 system has sparked a lot of interest in the scientific community because it is cheaper, faster, more precise, and more effective than existing genome editing technologies. CRISPR-Cas9 is a naturally occurring genome editing system that bacteria deploy as an immunological response. Bacteria that are virus-infected capture parts of the viruses' DNA and insert them into their own DNA in a specific pattern to form patterns known as CRISPR arrays. The CRISPR array’s pattern enables the bacteria to remember the viruses and associated proteins. In the event of a subsequent virus attack, the bacteria create RNA segments from CRISPR arrays that can recognize and bind to particular sections of the viral DNA. The bacteria then use Cas9 or a similar enzyme to cut the DNA apart, which disables the virus.
They generate a small RNA piece with a short "guide" sequence that connects (binds) to a particular target region in a cell's DNA, similar to the RNA segments bacteria produce from the CRISPR array. Additionally, this guide RNA binds to the Cas9 enzyme. When delivered into the specified cells, the guide RNA recognizes the desired DNA sequence and enables the Cas9 enzyme to cut an appropriate target site of the DNA, mimicking the process in bacteria.
The prevention and treatment of human diseases is a major area of concern for genome editing. Genome editing is currently employed in research facilities to study diseases in cells and animal models. Researchers are currently determining whether this approach is safe and efficient for usage in people. It is being investigated in research and clinical trials for a wide range of diseases, including hemophilia, cystic fibrosis, and sickle cell anemia. Additionally, it shows promising results in the management and prevention of complicated diseases like HIV infection, heart disease, cancer, and mental illness.
Mechanisms of CRISPR-Cas9 Genome Editing
The three phases of the CRISPR-Cas9 genome editing mechanism are recognition, cleavage, and repair. The intended sgRNA controls Cas-9 and, through its complementary base pair in the 5ʹcrRNA, recognises the target sequence in the gene of interest. In the absence of sgRNA, the Cas-9 protein remains dormant. Double-stranded breaks (DSBs) are produced by the Cas-9 nuclease at a location three base pairs upstream of Protospacer adjacent motif (PAM). PAM is a short (2–5 base-pair length) conserved DNA sequence located downstream of the cut site and its size varies depending on the type of bacteria. The Cas-9 protein, the most popular nuclease in genome editing tools, can identify the PAM sequence at 5-NGG-3 (N can be any nucleotide base). When Cas-9 locates a target site with the right PAM, it causes local DNA melting, which is followed by the synthesis of an RNA-DNA hybrid. However, the process by which the Cas-9 enzyme melts the target DNA sequence is still not fully known. The Cas-9 protein is then turned on to start cleaving DNA. Target DNA is broken down into its complementary and non-complementary strands by the HNH and RuvC domains, respectively, resulting primarily in blunt-ended double-strand breaks (DSBs). Finally, the host cellular machinery fixes the DSB.
Double-Stranded Break Repair Mechanisms
The two mechanisms used to repair the DSBs produced by the Cas-9 protein in the CRISPR-Cas9 process are non-homologous end joining (NHEJ) and homology-directed repair (HDR) pathways. NHEJ is active throughout the whole cell cycle and aids in the repair of DSBs by connecting DNA fragments through an enzymatic mechanism in the absence of exogenous homologous DNA. Although it is the most prevalent and effective cellular repair process, it is prone to mistakes and can produce minor random insertions or deletions (indels) at the cleavage site, which can result in frameshift mutations or premature stop codons. HDR requires the use of a homologous DNA template and is extremely precise. It is most active in the late S and G2 phases of the cell cycle. A significant number of donor (exogenous) DNA templates bearing a specific sequence are necessary for HDR in CRISPR gene editing. By inserting a donor DNA template with sequence homology at the anticipated DSB site, HDR carries out the precise gene insertion or replacement. (Figure 1)
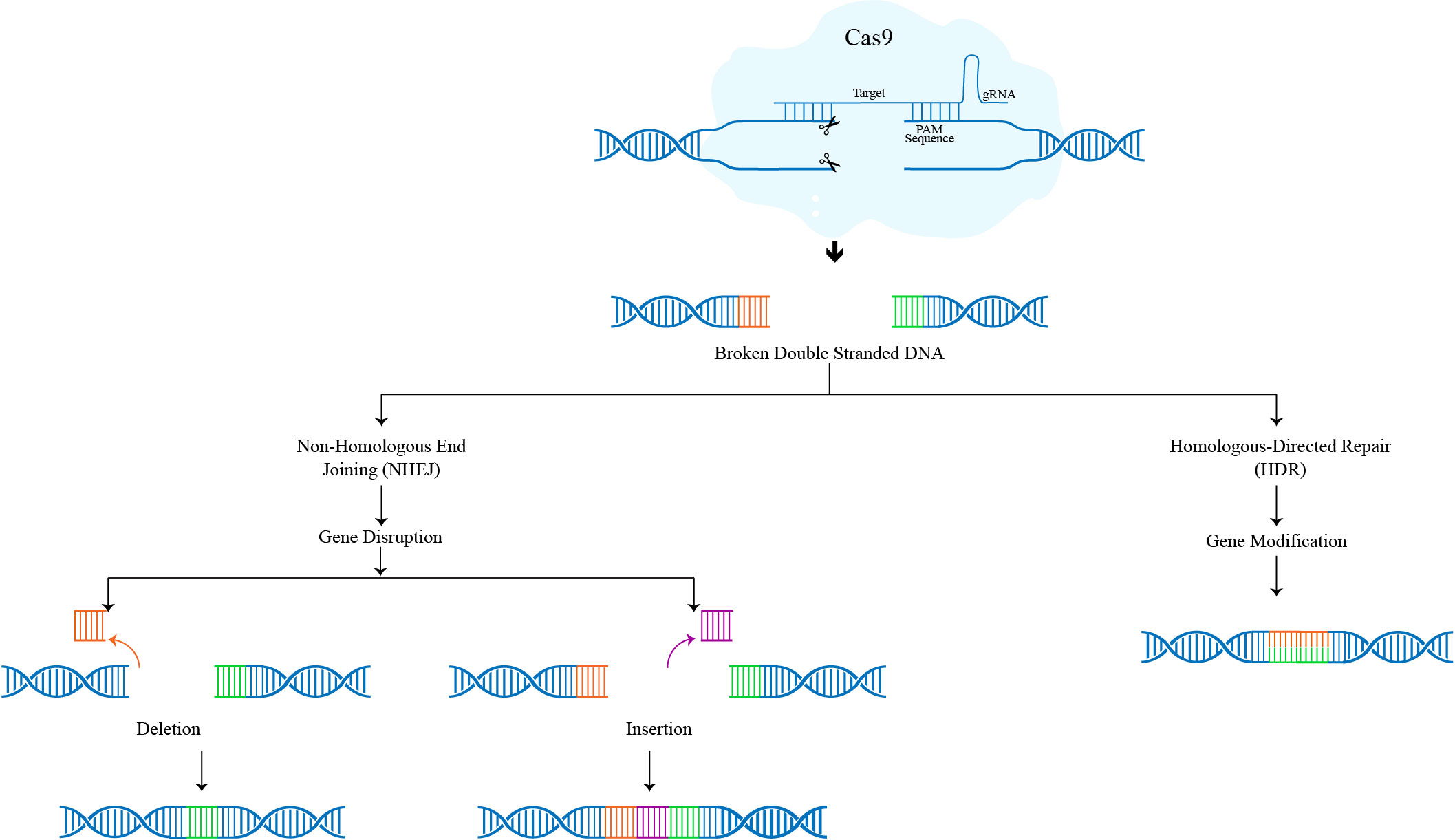
Figure 1: Mechanism of CRISPR-Cas9
Therapeutic Role of CRISPR-Cas-9
The first CRISPR-based therapy conducted on humans was to treat patients with refractory lung cancer. Firstly, T-cells were taken out from the patient’s blood and then modified in the lab using CRISPR-Cas9 to eliminate genes (PD-1, TRBC, and TRAC) that might interfere with the immune system's ability to kill cancer cells. The patients were then given the engineered T-cells, these cells have the ability to specifically target and destroy cancer cells. Furthermore, no side effects were reported and these modified T-cells can be detected even after 6-8 months post-introduction. The use of CRISPR-Cas-9 gene-editing technology to treat infectious disorders brought on by bacteria is also possible. The treatment of HIV, the virus that causes AIDS, is one of the key research areas. In addition to focusing on the HIV genome, CRISPR-Cas9 technology can also be used to modify the host cells' chemokine co-receptor type-5 (CCR5) genes in order to prevent HIV from entering the cells. For instance, a study done in vitro in China found that CRISPR-Cas9 genome editing of CCR5 did not cause any cell toxicity (infection), and the researchers came to the conclusion that edited cells were more successful at preventing HIV infection than unmodified cells.
Role in Agriculture
As the global population rises, the risk of agricultural resource scarcity is real. New technologies are therefore required to increase and improve natural food production. CRISPR-Cas-9 an existing addition to the field has been used to genetically modify to boost their drought tolerance, nutritional value, disease resistance, and shelf life. The three major ways that CRISPR is addressing the global food crisis are: food supplies can be replenished, plants can survive in harsh environments, and their general health may be improved.
Role in Gene Activation and Silencing
CRISPR-Cas-9 can be employed for purposes other than genome editing, such as artificially regulating (activating or repressing) a specific gene target. By deactivating the HNH and RuvC domains of Cas-9 endonuclease, researchers created dCas-9 nuclease, an advanced modified Cas-9 endonuclease. The dCas-9 nuclease is devoid of DNA cleavage activity, but it retains DNA binding activity. To create the CRISPR/dCas-9 complex, transcriptional activators or inhibitors can be combined with dCas-9. In order to activate (CRISPRa) or mute (CRISPRi) the expression of a particular gene of interest, catalytically inactive dCas-9 might be utilised. By combining a marker, such as Green Fluorescent Proteins (GFP) with dCas-9 enzyme, CRISPR-dCas-9 can also be used to identify and see the precise location of the gene of interest within the cell (subcellular localization). This makes it possible to use endogenous loci in living cells for site-specific labelling and imaging.
Role in Gene Therapy
More than 6000 genetic disorders have been identified so far. However, the majority of the disorders lack efficient treatment. Gene therapy is the procedure of replacing the damaged gene with exogenous DNA and editing the mutant gene in its natural site. It is the most recent advancement in the medical biotechnology revolution. 22 gene therapy, including the cutting-edge CRISPR-Cas9 technology, have been approved for the treatment of human diseases since 1998. Since its discovery in 2012, CRISPR-Cas9 gene editing has offered hope for the treatment of the majority of genetic disorders, including sickle cell disease, cystic fibrosis, β-thalassemia, and muscular dystrophy. Clinical trials have also used CRISPR-Cas9 for targeted sickle cell disease (SCD) therapy and -thalassemia treatment. Red blood cells with SCD have an autosomal recessive genetic disorder that results from a point mutation in the β-globin chain of hemoglobin, causing sickle haemoglobin (HbS). HbS polymerization causes serious clinical consequences such hemolytic anaemia during the deoxygenation process. The two primary ways that CRISPR-Cas-9 is being used to treat SCD are either direct gene repair of the haemoglobin S gene or increasing foetal -globin. However, increasing foetal haemoglobin is the way that is most frequently employed in clinical trials. Patients' bone marrow cells are first extracted, and then CRISPR/Cas-9 is used to inhibit the B-cell lymphoma 11A (BCL11A) gene, which prevents the formation of foetal haemoglobin. The gene-edited cells are then reintroduced into the body. On chromosome 2, the 200-base pair BCL11A gene is important for switching β -globin into the β -globin chain by suppressing the production of the β -globin gene. The severity and symptoms of SCD will be lessened once this gene is inhibited using CRISPR-Cas9 because more foetal haemoglobin containing -globin will be produced in the red blood cells. Additionally, researchers are looking into CRISPR-Cas-9 as a cystic fibrosis treatment. Cystic fibrosis is caused by a genetic mutation in the CFTR gene, which reduces the protein's structural stability and ability to perform its intended function. The apical surface of the epithelial cells of the lung, colon, pancreas, and reproductive tract contains the CFTR protein, an anion channel protein controlled by protein kinase-A. Symptom-based therapy (including antibiotics, bronchodilators, and mucus-thinning medications) and CFTR modifying pharmaceuticals have emerged as the first-line treatments for cystic fibrosis even though there is no known cure. These therapies help patients relieve symptoms and lower their risk of complications.
In 2013, scientists used intestinal stem cells from two cystic fibrosis patients and fixed the CFTR locus mutation, leading to the production of the correct gene and complete functionality of the protein. Since then, the effectiveness of CRISPR-Cas-9 technology in treating cystic fibrosis has been established. Furthermore, CRISPR-Cas-9 has proved effective in treating patient-induced pluripotent stem cells for Duchenne muscular dystrophy (DMD), which is characterised by muscle weakness and brought on by a mutation in the dystrophin gene. Despite significant efforts, the present DMD treatment is still supportive rather than curative. Exon skipping, cell therapy, and gene therapy are among the treatment modalities currently being researched to restore dystrophin expression in DMD muscles. The innovative and promising methods for repairing the DMD gene, which restores the expression of the dystrophin protein, include the deletion/excision of intragenic DNA and the removal of the duplicated exon by CRISPR-Cas-9. Furthermore, the most recent studies demonstrate that the single-base editing and prime editing systems enabled by CRISPR-Cas can introduce mutations into cellular DNA without the need for a donor template. The potential of indels that differ from those produced by standard Cas-9 is decreased because the CRISPR-Cas-base editor and prime editor system do not induce DSB. Adenine base editor (ABE) and cytosine base editor (CBE) are the two types of base editors that have been created so far (ABE). The CBE is a specific kind of base editor made of cytidine deaminase coupled with dead or insufficiently catalytic Cas-9 (dCas-9). It is a cutting-edge gene therapy technique that is capable of producing precise base alterations from cytidine (C) to thymidine (T). PAM sequences featuring G, T, or A bases continue to limit the CBE base editor's target range. A more sophisticated base editor with increased PAM compatibility for cytidine dinucleotides, known as nNme2-CBE (found from Neisseria meningitides), has recently been created in both human cells and rabbit embryos. The base-pair alteration from adenosine (A) to guanosine is corrected by the ABE using adenosine deaminase attached to dCas-9 (G). Overall, single-base editing of point mutations using dCas-9 fused to cytidine deaminase or adenosine deaminase is a secure and effective technique. But only four-transition mutations can be corrected by either base editor (purine to purine or pyrimidine to pyrimidine).
To overcome this shortcoming, the most recent member of the CRISPR genome editing toolkit called Prime Editor (PE) has been developed to extend the scope of DNA editing beyond the four types of transition mutations. The Cas-9 nickase cuts the non-complementary strand of DNA three bases upstream from the PAM site when the pegRNA detects the target nucleotide sequence, revealing a 3'-OH nick of genomic DNA. The 3' nick is then extended by the reverse transcriptase by duplicating the edit sequence of the pegRNA. Therefore, PE corrects minor insertion and deletion mutations in genetic disorders in addition to all 12 potential base-to-base transitions and transversion mutations.
BPS Bioscience Inc. with custom cell line development services, generate custom knock-out and knock-in cell lines using CRISPR-Cas9 licensed technology. The development process is comprised of five milestones where data is provided after each milestone completion. BPS Bioscience Inc. offers over 70 cell types across cancerous and noncancerous cell lines (https://bpsbioscience.com ).
Five steps of knock-out cell line generation:
1. Molecular Biology
BPS Bioscience, Inc. will design and synthesize three short guide RNA (sgRNA) sequences corresponding to the gene of interest to be knocked-out, based on criteria to maximize cutting efficiency while minimizing potential off-targeting.
2. CRISPR Transfection
Depending on the cell-type, cells can be transfected via electroporation, liposome-based transfection, or viral infection. The parental cell line can be provided or supplied by BPS Bioscience, Inc.
3. Limiting Dilution
Based on the results of the initial pool testing, the cell pool will be clonally diluted and the single cell-derived clones will be expanded.
4. Screen for Either Loss of Expression or the Knock-In Mutation
The expression level of the gene of interest will be analysed via Western Blot or FACS.
5. Confirmation
Genes showing loss of expression of the gene of interest will be further analysed through genomic sequencing.
BTL Biotechno Labs Pvt. Ltd. is the distributor of BPS Bioscience Inc in India, supplying the CRISPR Cas 9 genome editing products to the scientific community. To explore the wide variety of life science products, follow the product section link https://biotechnolabs.com/products
For product details, please connect with us at info@biotechnolabs.com.